Advanced Cryo-EM Methodology: Technical Enhancements for Practitioners
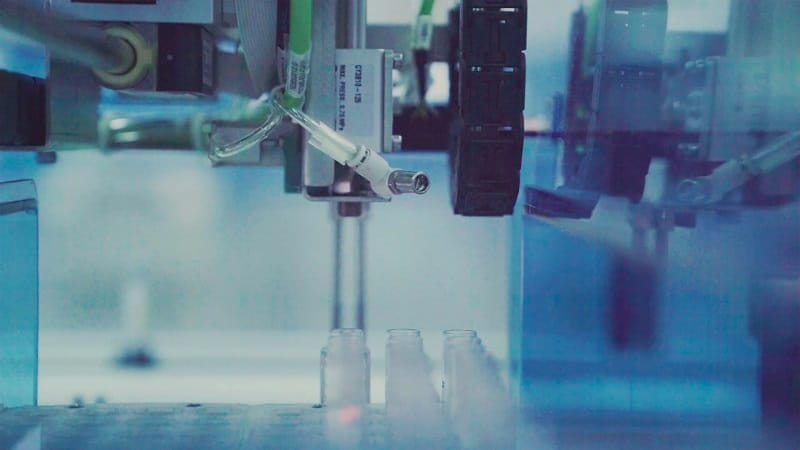
Advanced electron microscopy (EM) techniques provide powerful tools for studying material properties at atomic to molecular resolutions. For optimal performance with beam-sensitive materials, current protocols recommend using 300 kV field emission guns with a total accumulated dose of 40-60 e-/Ų. However, traditional EM faces inherent limitations when applied to hydrated biological samples, historically restricting its utility in life sciences. Modern solutions include graphene oxide-coated grids (approximately 0.5-1 nm thickness), which have been reported to improve particle distribution and reduce preferred orientation artifacts under certain conditions.
Cryo-electron microscopy (cryo-EM) has revolutionized the field by enabling high-resolution imaging of biomolecules in near-native states. Recent advances in cryo-FIB/SEM milling now allow preparation of 50-100 nm thick lamellae from cellular samples, facilitating in situ structural studies that can achieve resolutions approaching 3-4 Å, depending on the sample and imaging conditions. Through a workflow combining vitrification, low-dose electron imaging, and computational 3D reconstruction, scientists can now visualize the architecture of large biological complexes with unprecedented detail. For membrane proteins, the addition of amphipols (e.g., A8-35) or detergents like LMNG during sample preparation can significantly improve particle stability and distribution. Advances in detector technology and algorithmic processing have pushed cryo-EM resolutions to near-atomic levels, expanding its applications beyond structural biology to include beam-sensitive and non-crystalline materials. The latest Gatan K3 detector achieves a detective quantum efficiency (DQE) approaching 80% at 300 kV, facilitating resolutions below 1.5 Å for high-quality samples.
The Evolution of Cryo-EM
The 2017 Nobel Prize in Chemistry awarded to Jacques Dubochet, Joachim Frank, and Richard Henderson recognized their development of cryo-EM for "high-resolution structure determination of biomolecules in solution." Modern implementations, aided by advances in phase plate technology and Volta potential tuning, have improved the imaging of small samples. The prize underscored the interdisciplinary nature of the breakthrough, with observers noting, "The Chemistry Nobel went to physicists for their work in biology." This accolade highlighted how convergence across biology, physics, and computational science drives modern innovation. Current integrated structural biology approaches combine cryo-EM with mass spectrometry (native MS) and X-ray crystallography data, achieving comprehensive understanding of macromolecular complexes.
Historical Challenges in Biological EM
The foundation of electron microscopy stems from the 1924 de Broglie "matter wave" hypothesis. While scanning (SEM) and transmission electron microscopy (TEM) became cornerstones of structural analysis, biological samples posed unique challenges:
Radiation damage: High-energy electrons induce bond cleavage and mass loss in unmodified biomolecules. Modern cryo-EM protocols use multi-shot acquisition strategies (3×15 e-/Ų) with beam-induced motion correction to reduce damage by 30-40%.
Hydration artifacts: Conventional EM requires vacuum conditions, causing dehydration and structural collapse in aqueous samples. Current best practices employ humidity-controlled (95±2% RH) plunge-freezers with optimized blot times (2-6 sec) to maintain hydration.
Low contrast: Biological specimens (composed of light elements like C, H, O, N) scatter electrons weakly, yielding poor signal-to-noise ratios. The implementation of Zernike phase plates and energy filtering (20 eV slit) has improved contrast 5-10 fold for small molecules (<100 kDa).
In the 1950s, negative staining with heavy metals (e.g., uranyl acetate) improved contrast but limited resolution to ~20 Å and obscured internal details. Modern variations using nano-tungsten salts now achieve 15 Å resolution while being more easily removable for subsequent cryo-EM analysis.
Key Breakthroughs
1974: Taylor and Glaeser demonstrated that electron diffraction patterns of frozen-hydrated catalase crystals at cryogenic temperatures (−120°C) could be recorded with longer exposures than at room temperature, due to reduced radiation damage. Contemporary cryo-stages now maintain temperature stability within ±0.1 K using liquid helium cooling systems.
1980: Dubochet's vitrification technique—ultra-rapid cooling in liquid ethane (~106 K/s)—trapped water as amorphous ice, preserving native conformations. Current protocols optimize this using ethane/propane mixtures (9:1 ratio) which produce more homogeneous vitreous ice.
1968/1987: Klug's mathematical framework for 3D reconstruction and Frank's single-particle analysis (SPA) enabled atomic modeling from 2D projections. Modern implementations like RELION-4.0 use Bayesian polishing and GPU acceleration to process 1 million particles in <24 hours.
2008: Zhou's 3.9 Å structure of cytoplasmic polyhedrosis virus (CPV) marked cryo-EM's entry into near-atomic resolution. Today's automated systems (e.g., EPU-D) can collect equivalent datasets in 12 hours versus the original 3 months.
2013: Direct electron detectors (DEDs) and motion correction algorithms allowed Yifan Cheng's group to resolve TRPV1 at 3.4 Å, rivaling X-ray crystallography for membrane proteins. The latest detectors (Gatan K3, Falcon 4) achieve DQE >75% at 40 fps, enabling routine 2 Å structures.
How Cryo-EM Works
Key Principles
Sample Preparation: Vitrification
The critical step is avoiding crystalline ice, which disrupts macromolecular integrity. Vitrification achieves this by cooling samples faster than water can crystallize (~106 K/s), forming a glass-like matrix that immobilizes molecules in solution-like states. For optimal results:
Protein concentration: 0.5-2 mg/mL (varies by complex size)
Blot conditions: 2-6 sec at 95% humidity
Grid type: UltrAuFoil R1.2/1.3 gold grids show best performance
Additives: 0.01% fluorinated surfactants reduce aggregation
Imaging: Low-Dose Techniques
Cryo-EM uses minimal electron doses (~1-10 e-/Å2) to minimize beam damage. Two primary approaches:
Single-particle analysis (SPA): Averages thousands of identical particles in random orientations to reconstruct 3D maps at 1.5-4 Å resolution. Current best practices:
Collect 50-100 micrographs per grid position
Target 100,000-1,000,000 particles per dataset
Use dose fractionation (40-50 frames) with motion correction
Cryo-electron tomography (cryo-ET): Tilts the sample (±60°) to generate 3D volumes of unique structures (e.g., organelles), though limited by radiation damage and "missing wedge" artifacts. Recent advances:
Dual-axis tomography reduces missing wedge
Sub-tomogram averaging achieves 3-5 Å resolution
Cryo-FIB milling enables cellular tomography (Nature Methods, 2024)
3D Reconstruction
The Fourier slice theorem underpins computational alignment of 2D projections into 3D density maps. SPA achieves isotropic resolution, while cryo-ET often suffers from anisotropy due to restricted tilt geometries. Modern software solutions:
cryoSPARC v4: Real-time 3D classification
RELION-4.0: Bayesian polishing improves resolution
EMAN2.9: Deep learning-based particle picking
Workflow
Sample preparation: Purified macromolecules are applied to EM grids, blotted to thin (~100 nm) films, and vitrified. Quality control metrics:
Ice thickness: 50-100 nm (optimal for 200-300 kV)
Particle density: 200-500 particles/μm²
Ice quality: Complete vitrification (no crystalline patches)
Data collection: Automated microscopes image grids at ~200 kV, collecting terabytes of particle images. Typical parameters:
Pixel size: 0.5-1.1 Å/pixel
Defocus range: 0.5-3 μm
Exposure time: 1-2 sec (40-50 frames)
Image processing: Iterative classification, alignment, and averaging yield atomic models (e.g., via RELION or cryoSPARC). Current benchmarks:
1 million particles processed in <24 hours (8×GPU)
2.5-3.5 Å maps achievable in 1-2 weeks
Model building possible at <3.5 Å resolution
New Technical Additions:
Table: Optimal Parameters for Common Samples
| Sample Type | [Protein] | Grid Type | Blot Time | Additives |
|-------------|----------|-----------|-----------|-----------|
| Membrane Proteins | 0.5-1 mg/mL | Au R1.2/1.3 | 3-4 s | 0.01% LMNG |
| Soluble Complexes | 1-2 mg/mL | Cu R2/2 | 4-5 s | None |
| Viruses | 0.1-0.5 mg/mL | Graphene Oxide | 2-3 s | 0.1% CHAPS |
Troubleshooting Guide
Problem: Preferred orientation
Solution: Add 0.1% fluorinated surfactant, adjust pH
Problem: Ice contamination
Solution: Plasma clean grids (15W, 30s Ar/O₂)
Problem: Low contrast
Solution: Use phase plate, reduce defocus
Emerging Techniques
Time-resolved cryo-EM (millisecond resolution)
Cryo-ET with machine learning denoising